Most of the students interested in joining my lab do not come because they want to make measurements with small electrodes. They come because they want to solve big challenges – reducing the levels of carbon dioxide in the atmosphere, creating next-generation batteries that can store the electricity from growing solar and wind farms, putting renewable energies to good use in water remediation, or addressing the accumulation of polymers in the environment. However, understanding electrochemistry – a science at the interplay between chemistry and electricity – is central to tackling these challenges.
Electrochemistry is obviously not new – starting from Volta’s and Galvani’s experiments in the late 1700s, it continued developing alongside our ability to transduce chemical quantities into electric quantities, which is what we now do in most modern analysis. What is new and gives electrochemistry a new potential for change is the scale and the scope of its applications.
The scope? We have our growing appreciation for the potential of directly using electricity, primarily from emerging and rapidly growing renewable sources, to perform chemical reactions that were previously the domain of thermocatalysis or traditional synthesis. We now see emerging trends in using electrosynthesis for the production of hydrocarbon fuels, ammonia, hydrogen peroxide, and many specialty organic chemicals.
The scale? Well, that’s largely driven by the rapidly dropping costs of renewable electricity, which couples to societal needs – that go beyond small batteries for your cell phone. Now, we are ambitiously targeting transportation and storage for the electrical grid, as well as the chemical industry, which also looks towards decarbonization to accomplish goals set by environmental policy makers. Direct air capture of carbon dioxide, for instance, has implications at the scale of our “globe-sized beaker!”
The challenges described above are complex, but one thing is clear to us: It’s a great time to do electrochemistry – and that forms the basis of our group’s philosophy. My team works at the interface of analytical and materials chemistry (see image 1); in short, we develop new ways to look at electrochemical energy materials and interfaces. We also make use of materials concepts to develop new analytical methodologies. This interplay gives us great versatility to both figure out what happens with a material and come up with strategies and solutions to immediately address materials issues as we measure them. In a way, this is not a new strategy – our colleagues in disciplines such as materials science and engineering have been doing this for a while, using advanced instrumentation, such as X-ray and electron microscopy methods. What distinguishes us? Our ability to conduct chemical and reactivity measurements in situ and operando (in other words, in the proper electrochemical environments or during operation of the electrochemical devices) with high versatility in the laboratory, using spatially resolved (electro)chemistry tools. In that sense, we are closer to what many analytical scientists do.
A great example in our own laboratory is the development of nonaqueous flow batteries – a main collaborative project that our lab contributes to as part of the larger Joint Center for Energy Storage Research (JCESR, an energy innovation hub funded by the US Department of Energy). We are looking into ways to enable the use of energy storage in the form of charged molecules in organic electrolytes, which may afford them greater energy densities and versatility in structures than those allowed by aqueous electrolytes. One development from our end was the introduction of size-exclusion flow batteries, which involves using highly soluble redox polymers as charging media. Sitting at the interface of analytical and materials chemistry, we’ve been able to conceptualize the design of these materials, collaborate hand-in-hand with materials chemist to create more optimal molecules and battery devices, measure the electrochemical attributes of these molecules in different contexts from solutions to single particles, and then operate the devices while we monitored the activity using our in situ methods (see image 2).
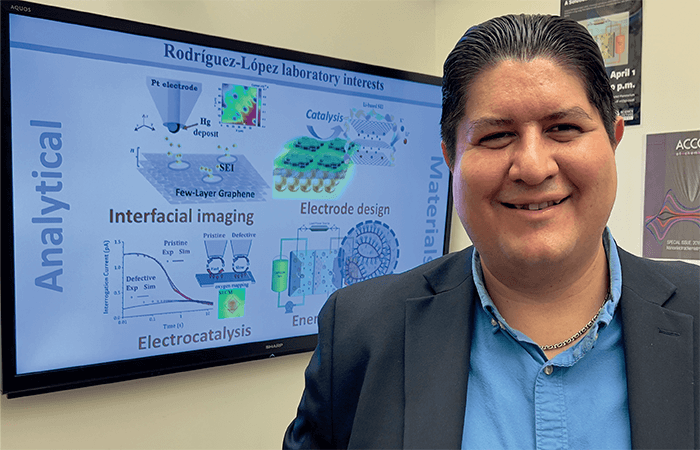
Big things in small packages
One interesting trend in the electrochemistry field is that, though the ambition of the field has grown in scale as previously discussed, progress in these areas is often driven by a reduction in the scale of the analyses themselves.
Certain inventions, such as the glass electrode used for measuring pH, and the development of popular techniques, such as cyclic voltammetry, were true game changers at the foundations of analytical chemistry in the 20th century. We now teach these concepts routinely at the undergraduate level. But when you combine these kinds of concepts with scanning probe microscopy, we’re able to learn about smaller-scale applications, such as battery interfaces, catalysts in action, or living biological entities. This integration happens under the umbrella of “electrochemical imaging,” and reminds me of the movie where the rat pleasantly combines the cheese and the strawberry (separately they are both good, but together they create a whole different concept). Similarly, we combine sensors with a scanning probe and advanced electrochemical techniques to evaluate the electrochemistry of small structures – whether cells, microorganisms, metal nanoparticles, individual polymer particles, or even features or adsorbates on a catalytic surface (see image 3).
There are many questions at this frontier. How do you measure the activity of a single catalytic site? How do you understand the complex processes undergoing a single particle of a Li-ion battery? How do you map the activity of a single synapse or a single droplet of an aerosol? These types of measurements distinguish themselves from others in that they capture the action of processes – not solely the structure. Measuring nanoscale phenomena and individual entities is one area where the electroanalytical community is highly active.
This trend is driven by developments in scanned probe techniques, the availability of nano-resolved scanners, and some maturity from the communities engaged in associated techniques (for example, scanning tunneling microscopy and atomic force microscopy). But in my field of (electro)chemical measurement and imaging, the main realization was that one could produce probes in many different formats to address reactivity in solution – from tiny hollow pipettes to small electrodes made of various materials to probes that can pick up a single particle.
Looking to the future, machine learning looks like a distinct game changer. Scientists working on scanned probe microscopy have started using these computational tools to perform automated measurements of materials in vacuum setups. Recent publications have showcased exciting strategies using advanced algorithms that are capable of reducing the complexity of some electrochemical measurements, including electrochemical imaging ones. Using such methodology, we can perform a variety of tasks – repeating otherwise dull routines with high precision or using advanced pattern recognition to decouple sample morphology from activity, for example. It is only a matter of time before these types of developments are used in a significant portion of labs.
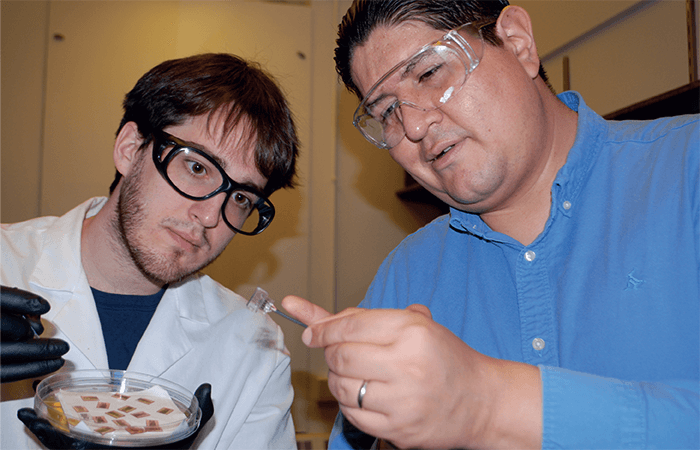
The big (and bigger) picture
I discussed the grand opportunities in using small probes to look at the action at electrodes for batteries or electrolyzers. But we also need to develop the ability to translate what we learn from these techniques to the actual devices – or to go from the in situ experiment to the operando experiment. Operando experiments, as outlined above, perform measurements in the actual device – for example, inside a battery, electrolyzer, or fuel cell. I think this is an area where analytical chemists – in partnership with many other disciplines including materials scientists, microscopists, physicists and most definitely engineers – have much to contribute. For example, analytical scientists could develop methods based on separations, spectroscopy, and diffractometry that speak to the needs of self-contained, closed systems that could be under pressure, electrochemical control, or in other difficult to probe environments.
These kinds of next-level measurements require two things: active collaboration/cooperation and education in electrochemistry. Collaboration implies a willingness to not only understand what the other is saying or doing, but also to have a proactive attitude to learn new things, strategies, and ways to shape working teams. An attitude of putting yourself in your collaborator’s shoes is also key for decreasing the “activation energy” for collaboration and for communication. I always tell my students that, regardless of the topic, my mission when attending any seminar/conference presentation is to actively answer a question: “How can my expertise and methods help this person in front of me?” I don’t always express these views to the speaker (and, when I do, it is typically in private), but it is always a useful exercise to think outside of your own technical and disciplinary boundaries.
The second ingredient is education in electrochemistry. I don’t like to assume that my collaborators understand what I am talking about, but I know that, if I give them the opportunity to learn more formally about my experiments, it could catalyze the collaboration process. In this context, my group has for the past 10 years been developing an experience that we call the “Electrochemistry Bootcamp.” In its current form, this program combines laboratory and classroom instruction over three days. The course includes six training modules (basic electrochemical setup, electrocatalysis, simulations, ultramicroelectrodes, batteries and preparative electrochemistry, and scanning electrochemical microscopy), with each one divided into three round-robin demonstrations and activities in the laboratory or computer. As a result, students are introduced to 15 different experiments, six lectures, and one simulation session that enables them to learn the vocabulary and practice of electrochemistry. For novice students, the course helps decrease anxiety around setting up and running experiments; for advanced students, it challenges their knowledge and fills gaps. We are only able to run the course about once every year (the last one had 45 participants) – but imagine if we all did something similar, actively, and transparently helping others learn more about our own fields… I think we could dramatically accelerate collaborations to tackle big problems. And there are plenty of big problems.
Tackling climate change, understanding cancer, exploring the complexity of the brain, using versatile chemical sensors to make more efficient agriculture, optimizing water use and remediation, controlling pollution, designing the self-driving lab of the future, using renewable electricity whenever possible in industrial processes, removing plastics from the ocean, and addressing how redox species impact our body functions…
There are many complex and urgent gaps in human knowledge that we could help fill with better interdisciplinary electrochemistry. But, rather than being overwhelmed, I am excited and positive about the future of my field.
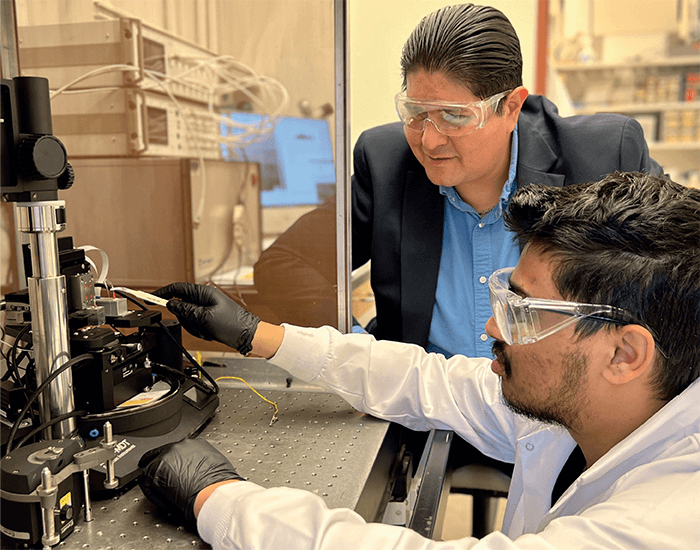
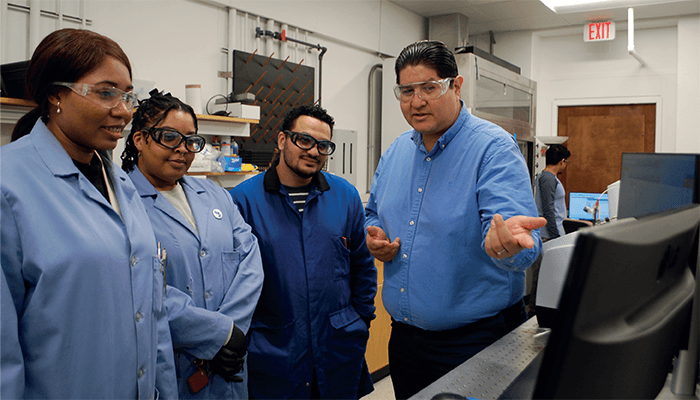
Our Piece of the Puzzle
Our group develops strategies to look at chemical and electrochemical processes at the surface of electrodes using small electrochemical probes positioned near the action. We use a technique called scanning electrochemical microscopy (SECM) – although there are many variations of this concept that can be used. Some of the techniques we have introduced include:
- a Hg microprobe that can look at Li+ and other ions as they are inserted and released from an operating Li-ion battery electrode
- the use of a redox-nanotitrations that can measure tiny amounts of surface-bound species (oxides, hydrides, and so on) at electrocatalysts to understand surface reaction mechanisms
- the use of redox-active spin traps to identify otherwise elusive reactive oxygen species right next to the electrode
- the use of nano electrodes that can touch individual redox-active polymer micro and nano particles intended for redox flow batteries so we can perform charge/discharge experiments on these tiny entities.
We can also use these electrochemical techniques to probe non-electrochemical systems. All these techniques rely on a small probe that can engage in highly versatile measurement and imaging experiments with its subject – a Li-ion battery, a flow battery, an electrocatalyst. Energy sciences are a big focus of our group, but many of these techniques can be easily extrapolated to other systems such as electrolyzers for chemical production or even living cells.
Automation towards the self-driving electrochemical experiment is another aspect that our laboratory is exploring. We are big believers in the use of small electrodes, which offer a powerful array of electroanalytical techniques in small volumes. They can help us more efficiently explore many materials, such as those produced from high throughput combinatorial experiments or more modest wet laboratories. For example, we have recently developed automated routines by combining Python programs and third-party libraries with microfabricated electrode chips that allow us to perform otherwise complex redox-active lifetime measurements with simplicity and high reproducibility. In another work, we simplified the staple experiment for any aspiring electrochemist – measuring the diffusion coefficient of a molecule – using automated commands via an application programming interface that acts as a translator of high-level functions for potentially several commercial potentiostats. In fact, we trimmed the time it took for an exert user to perform the experiment from ~30 min to 1 min 40 s of actual work, while presenting a rigorous electrochemical analysis. We are now looking closely into the idea of a self-driving electrochemistry laboratory for energy materials discovery.
Whatever the ultimate applications, we are really excited about increasing the sophistication of electrochemical experiments using these new computational tools.