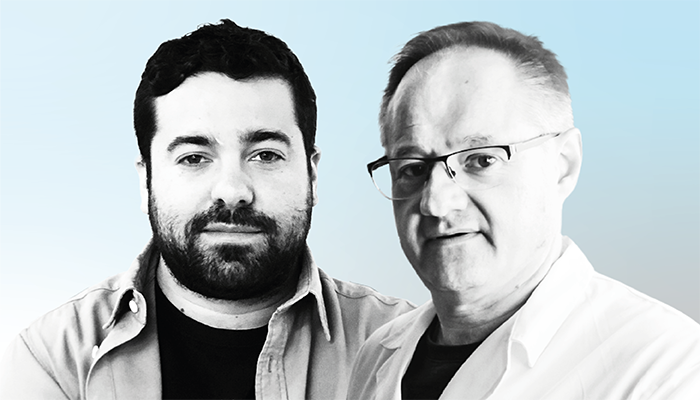
Credit: Supplied by Authors
3D printing has made tremendous strides over the last decade – across a diverse range of fields. And given the unique opportunities offered in terms of object reconfiguration, optimization and prototyping, 3D printing is opening new avenues in the realm of analytical science as well.
Today, 3D printing merely requires a digital model that is easily transferred as a “stl” file for accurate reproduction of the structure along with electronic access to a consumer-grade printer, thereby facilitating access to the model/printed object for potential users worldwide. Early developments were geared towards the affordable fabrication of millifluidic units without the need for specialized technical skills or sophisticated resources, such as clean-room facilities or photolithographic equipment. Now, 3D printing has evolved to the point of being employed for the manufacture and commercialization of fluidic devices for point-of-care analysis.
At this juncture, readers may be wondering whether 3D printing has something relevant to offer to the first stage of the analytical process, that is, sample handling and processing. We will seek to show that indeed it does – by shedding light on a selection of promising examples from across the three most commonly accepted printing technologies in the sample preparation domain: (i) fused deposition modeling (FDM), (ii) vat polymerization or stereolithography (SLA), and (iii) photopolymer inkjet printing (PIP). Although all of these techniques are based on the layer-by-layer approach of fabricating the final object, the methods of forming these layers differ significantly. Briefly, FDM forms each layer by extruding a thermoplastic at high temperature. SLA, despite having different fabrication modes, uses a photopolymerizable liquid resin with which the layers are formed. PIP employs a similar system to SLA, but uses wax as a filling support. In any case, readers are referred to a recent review article for insights into the printing process and the pros and cons of the varied printing technologies in the analytical chemistry arena (1).
The growing impact of FDM/SL/PIP in the sample preparation field is the result of recent advances in the variety of printable materials commercially available in terms of chemical functionality, including sustainable biomass-containing filaments for FDM, along with progress in making printers more reliable with higher resolution and lower minimum feature sizes. The current state-of-the-art of 3D printing in the design of sorptive materials and membrane-phases for microextraction ought to be broken down from two different perspectives, namely, (i) the purpose of the design and (ii) the material functionality, with distinct levels of complexity, in sample preparation workflows.
Firstly, from a design point of view, 3D printing offers the opportunity to move beyond traditional configurations by creating novel structures that enhance the sample preparation workflow without being directly involved in the extraction process (first level of complexity). In this context, the combination of innovative 3D-printed platforms with external sorptive materials and membrane phases might significantly improve the performance of the extraction methods. In this sense, the preparation of novel millifluidic systems for pressure-driven Lab-On-a-Valve methodologies combined with TiO2 beads or permeable membranes (2), or microflow injection systems with channels of square cross-section combined with magnetic materials (3), are nice examples of the use of 3D printing systems to produce customized holders, containers, and housing for handling sorptive phases in microextraction approaches.
From a material functionality point of view, the second level of complexity involves the use of commercially available raw SL material as the extraction phase itself, or multi-material FDM for all-in-one printing of a column holder and a polymeric extraction phase, e.g., using a polyvinylalcohol (PVA)-containing filament that is made porous after washing with water. Likewise, dialytic membranes can be prepared in-situ with PVA-containing PIP material, the porosity of which is strongly governed by the printing parameters, such as the orientation of the printed membrane. Intriguing applications at this level have been also accomplished with stimulus-responsive printable materials that change shape (following pH or temperature changes, for example) and structure – as part of a so-called 4D printing approach (4).
The third level of complexity relies on pre- or post-printing functionalization of the printable material to expand the functionality of the sorptive/extraction phase in a customized way. Examples of pre-functionalization involve mixing SL resins with metal or carbon nanomaterials for selective extraction, or in-house extruding distinct material components, including recycled oil, to confer new (electro)chemical, mechanical or catalytic (e.g., nanozyme-like) properties to the composite. This capability opens up a vast array of possibilities in analytical science (5,6). Post-printing functionalization encompasses exploiting the reactivity of the polymer after printing to either dynamically coat (physically entrapped or modified) or covalently attach new functional groups, to boost selective reactivity against target species. For example, 3D printing fosters the fabrication of tailor-made rotating systems with optimized geometry and covalently modified with selective functional moieties (e.g., antibodies) to facilitate preconcentration and reduce tedious steps of solid-phase extraction protocols, such as evaporation and re-constitution of extracts. (7)
The four level of complexity encompasses the fabrication of multi-material unibody devices with intricate/flexible architecture to accommodate the entire analytical procedure, that is, the injection of high matrix samples, such as biological specimens, which are then cleaned-up/extracted, either by integrated print-for-purpose membranes or sorptive cuboid-type/monolithic column structures, followed by elution/derivatization and/or microscale chromatographic/electrophoretic separation and, finally, on-site optical recording/visual monitoring of the target species (8).
In brief, opportunities and potentialities of 3D printing in the analytical sample preparation field are limited merely by the user’s imagination. We envisage that a vast number of current proof-of-concept applications of 3D printing, such as printable sorptive materials and membranes, will be easily transferred to the market in the near future. As a final note, interested readers are referred to a comprehensive monograph of the state-of-the-art of 3D printing in analytical chemistry co-edited by the authors (9) that will be published by the beginning of 2025.
Acknowledgments
The authors acknowledge financial support from the Spanish State Research Agency (Agencia Estatal de Investigación, AEI/10.13039/501100011033), the Spanish Ministry of Science, Innovation and Universities (Ministerio de Ciencia, Innovación y Universidades, MICIU) and the European Union (NextGenerationEU/PRTR) through projects PID2020-117686RB-C33 (AEI/MICIU) and TED2021-131303B-I00 (MICIU/AEI/NextGenerationEU/PRTR). Enrique Javier Carrasco-Correa acknowledges the funding of the project PID2021-125459OB-I00 from MICIU/AEI/NextGenerationEU/PRTR and MFA/2022/034, INVEST/2022/425 and CIAICO/2022/183 from Generalitat Valenciana. The authors extend their appreciation to MICIU for granting the Spanish Network of Excellence on Sustainable sample preparation (RED2022-134079-T). This article is prepared in the framework of the Sample Preparation Study Group and Network, supported by the Division of Analytical Chemistry of the European Chemical Society (DAC-EuChemS).
References
- E. J. Carrasco-Correa et al, “The emerging role of 3D printing in the fabrication of detection systems,” TrAC-Trends Anal Chem, 136, 116177 (2021). DOI: 10.1016/j.trac.2020.116177.
- D. J. Cocovi-Solberg et al., “3D Printing: The second dawn of lab-on-valve fluidic platforms for automatic (bio)chemical assays,” Anal Chem, 91, 1, 1140-1149 (2019). DOI: 10.1021/acs.analchem.8b04900.
- H. Wang et al., “3D-printed microflow injection analysis platform for online magnetic nanoparticle sorptive extraction of antimicrobials in biological specimens as a front end to liquid chromatographic assays,” Anal Chem, 89, 22, 12541-12549 (2017). DOI: 10.1021/acs.analchem.7b03767.
- C.-Y. Wu et al., “4D-printed temperature-controlled flow-actuated solid-phase extraction devices,” Anal Chem, 93, 11497-11505 (2021). DOI: 10.1021/acs.analchem.1c01703.
- B. Wu et al., “Direct Conversion of McDonald’s Waste Cooking Oil into a Biodegradable High-Resolution 3D-Printing Resin,” ACS Sustain Chem Eng, 8, 2, 1171-1177 (2020). DOI: 10.1021/acssuschemeng.9b06281.
- P. Veteška et al., “Novel composite filament usable in low-cost 3D printers for fabrication of complex ceramic shapes,” App Mater Today, 22, 100949 (2021). DOI: 10.1016/j.apmt.2021.100949.
- E. J. Carrasco-Correa et al, “3D printed spinning cup-shaped device for immunoaffinity solid-phase extraction of diclofenac in wastewaters,” Microchim Acta, 189, 173 (2022). DOI: 10.1007/s00604-022-05267-9.
- F. Li et al., “Multimaterial 3D printed fluidic device for measuring pharmaceuticals in biological fluids,” Anal Chem, 91, 1758-1763 (2019). DOI: 10.1021/acs.analchem.8b03772.
-
J.M. Herrero-Martínez at al., “3D-printing in Analytical Chemistry,” Elsevier, The Netherlands, 2025, in press.