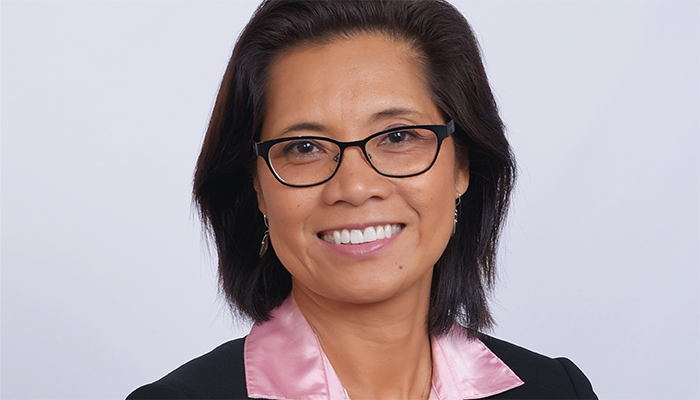
I first heard about PFAS in 2002, early in my academic career at the University at Buffalo. At that time, a visiting professor from the University of Toronto, Scott Mabury, introduced me to the complexity of analyzing these compounds and highlighted their widespread presence in air, water, and the environment. PFAS are notoriously difficult to detect, and this complexity initially deterred me from working on them. I was an assistant professor, focused on securing tenure, and I didn’t want to risk developing a method that might not work. So, for years, I avoided studying PFAS.
Fast forward to about five years ago, PFAS resurfaced in my professional life when collaborators started asking if I had a method for detecting them. They needed it badly, and that pushed me to dive deeper into the field.
Detection remains a central challenge, but it also underpins our response to the PFAS problem, whether it be monitoring or regulation. My PFAS research focuses on potential solutions and detection techniques. In assessing remediation technologies, detection plays a crucial role.
When you’re dealing with remediation, you really need to know where the PFAS are going and if they’ve been fully destroyed. For example, with biosolids or solids remediation techniques like incineration, pyrolysis, or gasification, even at high temperatures, you need to be sure whether you’re breaking them down completely or just volatilizing them. The same goes for groundwater treatments using plasma – you blast the PFAS, but where do they go? Are they just broken down into smaller, potentially harmful compounds? You need accurate detection to know.
Though we’ve made progress in analytical capabilities, there’s still a lot of room for improvement, especially when it comes to volatile PFAS and unknown degradation products that emerge from these remediation technologies. I’d say that analytical challenges remain a significant hurdle even today.
Can we remove or destroy PFAS?
There are several remediation technologies for PFAS in development; the choice of technology depends on what you’re trying to remediate. Right now, biosolids are a big issue. Many wastewater treatment plants used to apply biosolids on agricultural fields, but they’re now discovering that these biosolids often contain PFAS, especially if the treatment plant receives wastewater from an industrial source. There are farms in places like Maine that had to close due to high PFAS levels leaching from biosolids, which can contaminate surface water and groundwater, especially when it rains.
To address this issue, people are exploring pyrolysis or gasification to remove PFAS from biosolids. These processes essentially involve burning the material at very high temperatures, around 900–2,200 °F, to produce biochar or other byproducts (for example, syngas), depending on factors like oxygen levels and temperature. However, the challenge is that we don’t always know if PFAS are being fully destroyed or just volatilized during the process.
In our work, we’ve collaborated with engineering firms to analyze treated biosolids, such as biochar and condensates. We’re finding significantly reduced PFAS levels, but the remaining detected PFAS tend to be shorter-chain compounds, suggesting that long-chain PFAS might be breaking down into shorter-chain versions. We’re also working with the EPA to capture gasses during these processes, which my lab currently doesn’t have the capability to do. Capturing and analyzing volatile PFAS is highly challenging.
Most EPA-approved methods focus on water-soluble PFAS, typically analyzed via liquid chromatography-mass spectrometry (LC-MS). But even with those methods, we’re limited to PFAS that ionize, particularly those with negative charges. Neutral PFAS and positively charged PFAS are often missed because most methods are set up for negative-mode ionization. So, there’s still a lot we’re not capturing.
We’ve noticed this limitation when analyzing highly concentrated samples with fluorine nuclear magnetic resonance (19F-NMR) spectroscopy. This technique gives us a picture of “Total PFAS” in the sample, but when we take those same samples and analyze them by LC-MS, we detect less than 20 percent. In other words, a significant portion of organofluorines remain undetected by LC-MS. Again, we have very little knowledge about the volatile PFAS because there’s no standard method to analyze them.
Several strategies are being explored to remove PFAS from contaminated soil and water environments. For drinking water, activated carbon and anion exchange resin are the most widely used sorbents to capture PFAS. These materials work well, but after PFAS capture, you need to regenerate them or destroy the concentrated PFAS in the spent media. In addition, when using activated carbon filters, a key challenge is knowing when the filter is saturated and thus needs to be replaced. Breakthrough (where PFAS, especially short-chain compounds, start leaching back into the drinking water because the activated carbon has reached its capacity) is not acceptable for water utility companies.
Right now, the typical process is to sample the filtered water on a regular basis for LC-MS analysis at a certified lab. But if there is a backlog, results can take days, or even weeks. To avoid the risk of delayed result reporting, water utilities preemptively replace the carbon filters after a set volume of water has been treated, but this approach can be expensive. Hence, it would be incredibly helpful to have a faster method for detecting low levels of PFAS, ideally something like a PFAS-selective online sensor that could provide real-time data. Unfortunately, sensors for detecting PFAS at such low levels do not yet exist.
A gargantuan challenge
The sheer number of PFAS is huge, which also presents analytical challenges. Depending on the definition you use, there are over 5,000 PFAS, and if you go by the European definition, that number could exceed 12,000. These range from short-chain PFAS with just two carbon atoms to long-chain ones with up to 16 carbons.
The first challenge is sample extraction. You have to decide whether to focus on long-chain or short-chain compounds, as they behave differently. Then, you have to account for positively charged, negatively charged, and neutral compounds. For example, the EPA method for drinking water uses anion exchanger, which biases the extraction toward negatively charged compounds. Many of us use solid-phase extraction, which can combine reverse-phase and ion-exchange techniques, but even that has limitations, especially when dealing with high concentrations, as compounds can break through the cartridges.
The second challenge is understanding how to extract and concentrate the volatile PFAS without significant losses. After extraction, you are faced with a third challenge: separation. Some PFAS don’t retain well in traditional reversed phase LC columns. The EPA method starts with C4 PFAS, but there are C3 and C2 compounds that are highly polar and often ignored in many analyses. To address this issue, we’re developing methods using supercritical fluid chromatography (SFC), which is better suited for these highly polar compounds. This is important because many remediation techniques break PFAS down into ultra short-chain and highly polar compounds, and we need to be able to detect and evaluate whether PFAS are fully mineralized into fluoride ions, or just transformed into short-chain compounds.
As I previously noted, another analytical tool we’re exploring is fluorine NMR spectroscopy. NMR is a powerful tool because it doesn’t require standards to quantify PFAS. It can provide absolute signals for fluorine atoms and help us determine the Total PFAS content in the sample without extensive clean-up steps. However, the limitation of NMR is that we need high concentrations to get reliable signals, so NMR is better suited for analyzing high-concentration samples, like PFAS contaminated biosolids or industrial wastes. NMR is not ideal for low-concentration samples, like drinking water; we can concentrate large volumes of samples, but it is time consuming.
Another technique that is increasingly being used is combustion ion chromatography or CIC. This technique works well for determining total fluorine in solids – you combust the sample and measure the fluoride ions released. However, the method has limited selectivity; it can tell you there’s fluoride, but it won’t distinguish whether it came from PFAS or other fluorine-containing chemicals, like fluorinated pesticides. In contrast, 19F-NMR can be very selective because the fluorine signals in the spectra have specific chemical shifts that can be attributed to their locations in the molecule, which facilitates structural elucidation and chemical identification.
In truth, all of these techniques are necessary; often, we need a combination of approaches to get a comprehensive understanding of PFAS contamination and degradation.
Searching for a complete solution
To truly address the PFAS problem, everyone needs to work together, including regulatory authorities. We are still quite far from a complete solution. The more we regulate PFAS, the less they will enter the environment. However, the PFAS that are already in the environment will persist – they’re called “forever chemicals” for a reason. So, while regulatory measures are essential, we also need to focus on how to remediate what’s already there.
A major hurdle linked to regulation is the lack of toxicity data for many PFAS. We have a good amount of data on PFOA and PFOS, the C8 compounds, but there’s limited toxicity information for many of the other PFAS. These compounds often occur in mixtures, so how do you regulate mixtures? It is a significant challenge.
I’d also like to see more progress on sustainable bioremediation technologies. For example, if we could find naturally occurring bacteria that are capable of breaking down PFAS into carbon, hydrogen, and fluorine, it would be the ultimate remediation technology. In this regard, there are some promising lab-scale isolates, but we still have a long way to go to scale-up these solutions to something that can be implemented in real-world environments. Investing more in bioremediation research would be a great step forward.
About 25 years ago, people were concerned about antibiotics and pharmaceuticals in discharges from wastewater treatment plants. Now, many cities have adopted advanced treatment processes following conventional activated sludge systems, such as ozonation and carbon filtration, resulting in very clean water. But we don’t have anything like that yet that works well for complete PFAS treatment in highly contaminated water. If we could develop something similar on a municipal scale, it would have a huge impact. If we could inoculate wastewater treatment plants with bacteria that can degrade PFAS, we could prevent these compounds from ending up in surface water, which is often used for drinking water. And if all PFAS are removed during wastewater treatment, the biosolids produced would also be free of PFAS, giving the agriculture industry a valuable and safe fertilizer. Right now, many farmers are hesitant to use biosolids, and they have been banned elsewhere.
Why interdisciplinary collaboration is key
We need analytical chemists and engineers to collaborate because the chemists have the tools and expertise to detect and analyze PFAS, while the engineers are developing the remediation methods. As I mentioned earlier, they go hand in hand, and both aspects are equally challenging.
In fact, I recently attended a workshop organized by Intel and the National Science Foundation, which brought together analytical chemists and engineers to tackle the issues of PFAS in the semiconductor industry. Right now, the semiconductor industry still relies on PFAS for certain processes, like etching and preparing semiconductors, and they don’t yet have substitutes for these chemicals.
Though the industry recognizes the potential hazards associated with PFAS, they’re looking to academia and researchers for help in detecting and removing them from the industry’s wastewater. The reality is that until they find a greener substitute, they will continue to use PFAS, as they are essential to semiconductor production and other technologies.
After the two-day workshop, everyone was saying, “We should have done this workshop a long time ago. We need more of these types of gatherings for academic and industrial researchers to discuss challenges and opportunities so that we can find solutions to pressing problems more effectively by working collaboratively.” As academics, we often don’t know what’s happening in the industry or what the real-world problems are. On leaving that workshop, I had a much better idea on how to prioritize my research related to PFAS analysis.
As we continue to grapple with the complex issue of PFAS, it is clear that there is no single solution. Remediation technologies are advancing, but challenges in detection, degradation, and regulation remain.
To truly make strides, we need a multifaceted approach that includes stronger regulations, improved analytical techniques, innovative and cost-effective remediation technologies, sustainable substitutes, and greater interdisciplinary collaboration.
Image credit: Collage created using Adobe Stock images