Overtaking CARS
As a postdoc, I co-invented stimulated Raman scattering (SRS) microscopy for imaging chemical bonds (1). At that time, the Raman imaging field was dominated by coherent anti-Stokes Raman scattering (CARS) microscopy, but the high background and poor spectral fidelity of CARS meant there was a lot of “in-fighting” within the field. Rather than getting trapped in the CARS swamp, in 2008 I proposed SRS, another spectroscopic process for imaging. SRS quickly superseded CARS as the best method, and my cofirstauthored paper was cited more than 1,200 times.
The name’s bond
My work on novel microscopy led to my job at Columbia. At that time, label-free imaging was widely believed to be the major advantage of Raman microscopy, but I decided to challenge this paradigm by asking if one can introduce cleverly designed chemical bond-based probes. The pursuit of this answer not only changed my career but also led to significant technological innovations.
I identified a grand challenge: small biomolecules such as metabolites and drugs are extremely difficult to visualize inside cells. This is because bulky fluorescent tags will destroy native activities of small biomolecules. I soon realized that chemical-bond-based vibrational probes can be made as small as one chemical bond, and thus the native function of small biomolecules can be largely preserved. Subsequently, I developed a set of vibrational tags for SRS, including alkynes C=C (2) and stable isotopes such as 2H (3) and 13C (5). These tags are chemically inert and absent inside cells, hence the bioorthogonality. Moreover, they vibrate at unique frequencies in the cell-silent window, offering superb detection specificity.
In a body of publications, my group demonstrated a wide range of smallmolecule biochemistry in living cells, such as imaging drug trafficking, choline metabolism, glucose uptake, protein synthesis and degradation via amino acid, DNA replication and RNA turnover via nucleic acid. These applications are generating new biological insights; for example, my team discovered fatty-acidinduced liquid-solid phase separation in endoplasmic reticulum membranes as a biophysical basis for lipotoxicity (4). After many groups worldwide followed this approach, the work grew into a new field: bioorthogonal chemical imaging (6). The importance of small-molecule biochemistry in human health means that its impact is expanding beyond academia, with more pharma and biotech companies such as Merck and Pfizer are investing on this technology too.
Somewhere over the Carbow
Biological systems are inherently complex. The big challenge is the vast number of interacting players, ranging from protein networks to interacting organelles to synergistic cell types. Hence, simultaneous monitoring a large number of molecular species is indispensable for understanding the underlying complexity (7). However, due to the intrinsically broad fluorescent spectra (peak width around 50 nm), the prevalent optical microscopy can only image 2-5 targets at once (the so-called “color barrier”).
I realized that, compared with the broad fluorescence spectrum, Raman spectrum exhibits around 50 times narrower linewidth (approximately 1 nm), hence potentially offering many resolvable colors. My team designed a library of novel Raman dyes, the structures of which had never been reported, in which nitriles (C=N) are directly linked to the π conjugation system of chromophores. By meticulously modifying the chromophore scaffolds and doping 13C and 15N onto C=N, they fine-tuned the sharp Raman peaks to span 16-20 colors (8). Alongside spectroscopy, the team further developed electronic pre-resonant (epr) SRS microscopy. This 2017 paper in Nature is a true breakthrough on several fronts: (a) it breaks the fundamental “color barrier” for imaging complex systems; (b) it is the first systematic effort to engineer Raman dyes, while hundreds of labs have been engineering fluorescent dyes for decades; (c) with sensitivity approaching single molecules, epr-SRS itself is a breakthrough in spectroscopy (9).
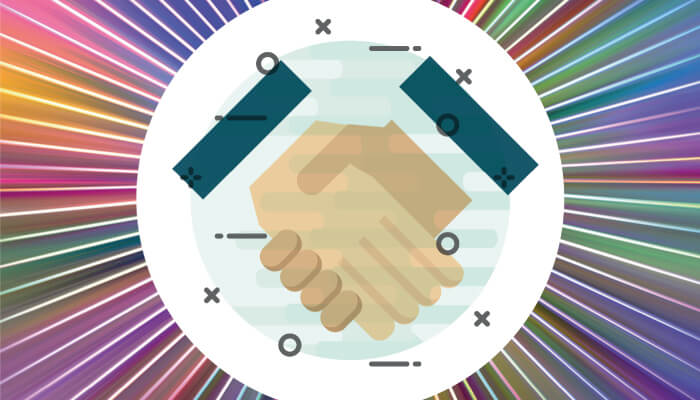
Recently they developed a new class of polyynes with 20 colors named “Carbon rainbow” (Carbow). They further functionalized Carbow and achieved 10-color optical imaging of cellular structures in living cells, which is the highest multiplexed imaging of specific targets inside living cells to date. Moreover, they achieved high-density optical data storage via combinatorial barcoding, which could transform highthroughput screening of drugs and cells (10). While this technique is being commercialized, many labs are already using it to unravel complex systems.
Getting a visual
Visualizing metabolism is essential to unraveling the mechanistic basis of many biological processes, but previous methods have limited resolution or biocompatibility. My team developed an imaging method (DO-SRS) that combines heavy water (D2O) probing and SRS to visualize metabolic dynamics in animals with high spatiotemporal resolution and molecular specificity (11), obtaining new biological insights such as myelination in developing brain and tumor heterogeneity. DOSRS is a powerful and universal method for studying metabolism and its heterogeneity, and my team (with collaborators) is employing DO-SRS to reveal previously unknown metabolic heterogeneity inside biofilm with insight into antibiotic resistance (12). DO-SRS also has clinical implications, as D2O is commonly used in humans too (13).
A battery of energy research
Visualization of ion transport in electrolytes provides fundamental understandings of electrolyte-electrode interactions. However, existing techniques find it hard to capture low ion concentrations and fast dynamics. Again, working with collaborators, my team showed that SRS microscopy is able to image ion transport in an operating lithium battery (14), which is the first application of SRS microscopy in the battery field. Their study provides critical insights into a long-lasting question: how Li+ concentration correlates to uneven lithium deposition. The ability to image ion transport in electrolytes with high sensitivity, speed and resolution will make a significant impact on broad fields of materials and energy research.
References
- CW Freudiger et al., Science, 322, 1857 (2008). L Wei et al., Nature Methods, 11, 410 (2014). L Wei et al., Proc Natl Acad Sci USA, 110, 11226 (2013). Y Shen et al., Proc Natl Acad Sci, 114, 13394 (2017). Y. Shen et al., Angew Chemie Int Ed Engl, 53, 5596 (2014). L Wei et al., Acc Chem Res, 49, 1494 (2016). JW Lichtman, W Denk, Science, 334, 618 (2011). L Wei et al., Nature, 544, 465 (2017). L Wei, W Min, J Phys Chem Lett, 9, 4294 (2018). F Hu et al., Nat Methods 15, 194 (2018). L Shi et al., Nat Commun, 9, 2995 (2018). K Schiessl et al., Nat Commun, 15, 10 (2019). PJ Jones, ST Leatherdale, Clin Sci, 80, 277 (1991). Q Cheng et al., Nat Commun, 9, 2942 (2018)